Heterojunction of ZnO@COF
A diagrammatic representation of the ZnO@COF Z-type heterojunction is displayed in Fig. 1a. ZnO nanospheres have been prepared through a solvothermal approach using Zn(OAc)2 ∙ 2H2O as the precursor38. Following this, ZnO nanospheres were treated with 3-aminopropyltriethoxysilane (APTES) to obtain amino-functionalized ZnO nanospheres (ZnO-APTES). In the next step, ZnO@COF core-shell hybrid materials with varying COF ratios were created by in situ growing COF (TP-BDOH, where TP stands for 2,4,6-triformylphloroglucinol and BDOH denotes 3,3’-dihydroxybenzidine) on ZnO-APTES via a Schiff base reaction. The morphologies of ZnO nanospheres, COF, and ZnO@COF were characterized utilizing scanning electron microscopy (SEM). The manufactured ZnO nanospheres exhibit a clear spherical shape with an average diameter of 150 ± 20 nm (Supplementary Fig. 1a). The synthesized COF demonstrates a fibrous network architecture composed of nanowires (Supplementary Fig. 1b). Analyzing the SEM images of ZnO@COF (Supplementary Fig. 1c–f), it is evident that ZnO nanospheres are uniformly enveloped by the fibrous COF. Evaluation via transmission electron microscopy (TEM) for both ZnO and ZnO@COF (Fig. 1b, c) additionally validates that ZnO nanoparticles are entirely covered with the COF layer, the thickness of which is approximately 20 nm, indicating a strong interaction between ZnO and COF24. Furthermore, the high-resolution TEM (HR-TEM) images of ZnO@COF reveal that two distinct crystal planes are in close proximity (Fig. 1d). The lattice spacings measure 0.28 nm and 0.26 nm, correlating to the (1 0 0) planes of ZnO and COF respectively, confirming the successful formation of the heterostructure. Elemental mapping images (EDS) indicate that C, N, O, and Zn are densely present on the surface of ZnO@COF, further substantiating the uniform encapsulation of ZnO by COF (Supplementary Fig. 2). The thermal stability of the fabricated ZnO, COF, and ZnO@COF was assessed using thermogravimetric analysis (TGA), exhibiting excellent thermal stability up to 320 °C under N2 atmosphere (Supplementary Fig. 3).
a Synthesis of ZnO, COF, and ZnO@COF (TP: 2,4,6-triformylphloroglucinol; BDOH: 3,3’-dihydroxybenzidine; APTES: 3-aminopropyltriethoxysilane). TEM images of b ZnO and c ZnO@COF. d HR-TEM image of ZnO@COF.
In order to accurately evaluate the characteristics of the materials, a series of heterojunctions named ZnO@COF-x have been synthesized by varying the COF ratio (x signifies the percentage of COF). The crystal structure and crystallinity of the synthesized samples were examined using powder X-ray diffraction (PXRD). The primary diffraction peak of COF at 3.45° corresponds to the (1 0 0) crystalline plane (Supplementary Fig. 4), aligning with the simulated results and confirming the creation of a long-range ordered crystal structure43. ZnO nanospheres demonstrate a hexagonal wurtzite phase (PDF 89-1397), with the primary diffraction peaks appearing at 31.74°, 34.38°, and 36.21° corresponding to the (1 0 0), (0 0 2), and (1 0 1) crystal planes, respectively (Supplementary Fig. 5). A series of distinctive characteristic diffraction peaks corresponding to both ZnO and COF can be observed in the XRD patterns of ZnO@COF, indicating the successful integration of the heterojunctions (Fig. 2a). Additionally, the coexistence of ZnO and COF in the Fourier-transform infrared (FT-IR) spectra of ZnO@COF further verifies that COF has been effectively grafted onto the ZnO surface via a Schiff base reaction (Supplementary Figs. 6 and 7).
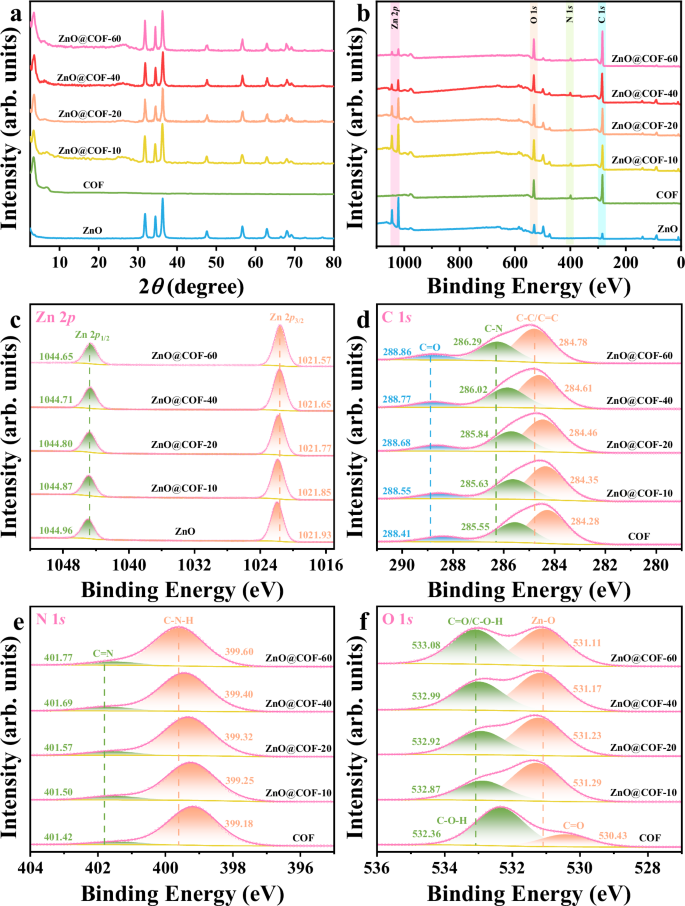
a PXRD patterns, b XPS spectra survey, c Zn 2p, d C 1 s, e N 1 s, f O 1 s of ZnO, COF, and ZnO@COF (c-f are XPS results of regions from the survey in b). The patterns of Fig. 2 are stacked. Source data for Fig. 2 are provided as a Source Data file.
To investigate the surface composition and chemical interactions within the ZnO@COF heterojunctions, X-ray photoelectron spectroscopy (XPS) characterization was performed. The XPS analyses confirm the existence of C, N, O, and Zn elements throughout the ZnO@COF heterojunctions, further supporting the successful synthesis of the composites (Fig. 2b). Notably, a shift towards lower binding energy for the Zn 2p core state is observed in the ZnO@COF heterojunction when compared to pure ZnO (Fig. 2c). This shift indicates an increase in the electron cloud density surrounding ZnO, suggesting improved electronic interactions within the heterojunction44. Conversely, the C 1 s and N 1 s peaks in ZnO@COF are displaced to higher energy levels compared to those in pure COF (Fig. 2d, e), indicating a reduction in electron cloud density around COF, further emphasizing the significant alterations within the electronic environment of the heterojunctions45.
The O 1 s spectra are categorized into two primary peaks: C = O/C-O-H and Zn-O (Fig. 2f). The peaks for C = O/C-O-H and Zn-O are shifted to higher and lower energy ranges respectively, consistent with the aforementioned results and becoming more pronounced with an increase in COF content. These intricate spectral modifications not only indicate a strong interaction between ZnO and COF, but also suggest a directionally induced migration of electrons from COF to ZnO due to close contact at the interface, consequently forming an effective internal electric field within the heterojunction21. This developed internal electric field is crucial for enhancing charge carrier separation and migration within the ZnO@COF heterojunction, with its efficacy significantly augmented by increasing COF content.
Piezoelectric properties of ZnO@COF
The piezoelectric effect is prompted by the mechanical stress applied on piezoelectric crystals, resulting in the generation of internal electric fields that drive various catalytic processes. The corresponding morphology of the samples was characterized through atomic force microscopy (AFM). The AFM images of ZnO and ZnO@COF indicate that they possess similar morphologies (Fig. 3a, b), with the particles of ZnO@COF being slightly thicker than those of ZnO by approximately 20 nm. The local piezoelectric response of the samples was assessed using Kelvin probe force microscopy (KPFM). Figures 3c, d present the surface potential images of ZnO and ZnO@COF within a 5 × 5 μm2 view field, illustrating theirpiezoelectric response characteristics. Under stress on the probe tip, ZnO@COF generates an internal electric field and produces a positive surface voltage of up to 71.57 mV, significantly surpassing that of ZnO (1.71 mV) (Fig. 3e, f). Piezoresponse force microscopy (PFM) was utilized to measure the piezoelectric response of the materials. The corresponding amplitudes and phase images of ZnO and ZnO@COF within the visible area of 5 × 5 µm2 are presented in Supplementary Figs. 8 and 9, respectively. As illustrated in Fig. 3g and h, the piezoelectric hysteresis curves for ZnO and ZnO@COF display a typical butterfly shape under ± 10 V DC bias electric field, with the phase angle reversed by 180°. The piezoelectric constant (d33) values for ZnO and ZnO@COF are 10.4 pm V−1 and 48.1 pm V−1, respectively, confirming the enhanced piezoelectric property exhibited by ZnO@COF. This observation is attributed to the improved polarization of the heterojunction structure ZnO@COF, which augments the piezoelectric potential response at the interface. The notable increase in piezoelectric current density for ZnO@COF compared to ZnO and COF (Fig. 3i) further supports the notion of superior piezoelectric characteristics following the formation of the heterojunction between ZnO and COF. It is important to highlight that as the COF content in the heterojunction increases, the piezoelectric performance also correspondingly enhances. This trend can be explained by the strengthening of the direct interaction force between ZnO and COF, resulting in improved electron transfer efficiency. However, excessive COF content can create space for electrons and holes to recombine, leading to a decrease in the piezoelectric performance of the heterojunction24.
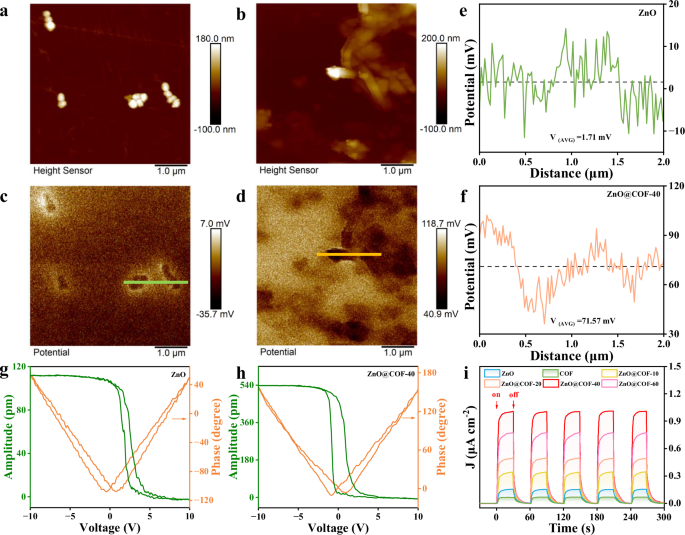
The topographic AFM (atomic force microscopy) images of a ZnO and b ZnO@COF. The surface KPFM (kelvin probe force microscopy) potential images of c ZnO and d ZnO@COF. Surface piezoelectric potentials of e ZnO and f ZnO@COF (e and f are the linescans from c and d). Piezoresponse amplitude curve and phase curve of g ZnO and h ZnO@COF. i Transient piezoelectric current response of ZnO, COF, and ZnO@COF. Source data for Fig. 3 are provided as a Source Data file.
To gain insights into the fundamental mechanisms underlying the enhanced piezoelectric performance of the heterojunction, the electronic property of ZnO, COF, and ZnO@COF heterojunction was analyzed. Electrochemical impedance spectroscopy (EIS) results reveal that ZnO@COF-40 has a smaller semicircular Nyquist plot radius compared to ZnO and COF (Fig. 4a), indicating reduced electron transfer resistance. This evidence points out that the inclusion of COFs with π-conjugated frameworks within ZnO generates an internal electric field at the interface, resulting in substantial polarization charges along the edges of ZnO. This diminishes the shielding effect of ZnO’s metallic-edge states, fostering efficient charge transfer and enhancing electrical conductivity. In steady-state photoluminescence (PL) spectra, the PL peaks for ZnO, COF, and ZnO@COF are centered at 480 nm (Fig. 4b). The peak intensity of ZnO@COF-40 is notably lowered, suggesting suppression of carrier recombination in ZnO@COF-40. Simultaneously, time-resolved photoluminescence (TRPL) spectra were employed to characterize the lifetimes of samples. As demonstrated in Fig. 4c, the fluorescence lifetimes of ZnO@COF composites exceed those of ZnO and COF, and improve with increased COF content until a slight decrease occurs when the COF percentage reaches 60. This can be attributed to the higher COF content facilitating carrier transport, thereby preventing recombination of holes and electrons. However, when COF content is excessively high, some electrons and holes may recombine on the surface of COF, leading to diminished piezoelectric performance.
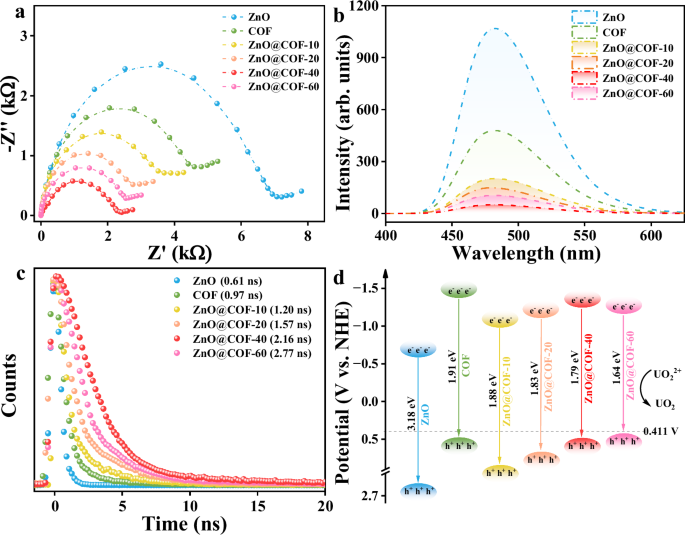
a Nyquist plots, b PL spectra, c TRPL spectra, d Band-structure diagram of ZnO, COF, and ZnO@COF. Source data for Fig. 4 are provided as a Source Data file.
The optical absorption properties and electronic band structures of the samples were examined using diffuse reflectance spectroscopy (DRS) and Mott-Schottky (M-S) spectroscopy. As indicated in Supplementary Fig. 10, all samples exhibit strong visible light absorption. Based on Kubelka-Munk calculations, the bandgaps for ZnO, COF, ZnO@COF-10, ZnO@COF-20, ZnO@COF-40, and ZnO@COF-60 are determined to be 3.18 eV, 1.91 eV, 1.88 eV, 1.83 eV, 1.79 eV, and 1.64 eV, respectively. It is observed that the bandgap of the composite materials gradually decreases with increasing COF content. Coupled with the Mott-Schottky test results, it is evident that the band structure of the catalysts aligns effectively with the equilibrium potential needed for uranium reduction (Supplementary Fig. 11). Notably, the conduction band position of ZnO@COF-60 is close to the equilibrium potential for uranium reduction (Fig. 4d). However, piezoelectric materials effectively modulate the potential barrier of the material’s electron band, which may lead ZnO@COF-60 to fail in satisfying the equilibrium potential of uranium reduction, thereby diminishing its catalytic efficacy.
As anticipated, the introduction of porous COF enhances the specific surface area and pore volume of the heterojunction, facilitating the adsorption of target molecules and providing additional active sites for the piezoelectric catalytic process. The surface area and porosity of COF and the best-performing ZnO@COF-40 were evaluated through nitrogen adsorption-desorption isotherms based on the Brunauer-Emmett-Teller (BET) model. Both COF and ZnO@COF-40 demonstrate similar adsorption isotherms, which are typical type I isotherms, indicating their microporous characteristics (Supplementary Fig. 12). The specific surface area of ZnO@COF-40 is 319.22 m2 g−1, which is slightly lower than that of COF (377.73 m2 g−1), yet still retains the intrinsic properties of COF. According to non-local density functional theory (NLDFT), the pore size distribution of COF and ZnO@COF-40 is concentrated around 1.41 nm (Supplementary Fig. 13). Therefore, the composite of ZnO@COF-40 exhibits remarkable piezoelectric catalytic characteristics while maintaining a high specific surface area, indicating its excellent potential as an effective catalyst.
Piezocatalytic extraction of uranium
The adaptability of ZnO@COF-40 to extract 100 ppm U(VI) through piezoelectric catalysis was assessed using ultrasonic treatment at 40 kHz and 120 W without requiring any organic sacrificial agents. As illustrated in Fig. 5a and Supplementary Fig. 14, due to the shielding effect of metal edge states, the effective piezoelectric catalytic removal rate of ZnO is only 11.57%. COF exhibits a minimal piezoelectric uranium reduction rate of 19.72%, attributed to the rapid recombination of electron-hole pairs. In contrast, the physical combination of ZnO and COF still shows a lower uranium removal efficiency of 16.73%; however, the piezoelectric catalytic activity of the composite materials is significantly improved. Among these, ZnO@COF-40 demonstrates the fastest adsorption kinetics, eliminating 95.25% of 100 ppm U within 1 minute, following a pseudo-second-order model (Supplementary Fig. 15 and Supplementary Table 1). This phenomenon can be attributed to the creation of an internal electric field between ZnO and COF, which effectively counteracts the shielding effect of ZnO metal edge states. The generated piezoelectric internal electric field can effectively modulate charge carrier transport and diminish the rapid recombination of electron-hole pairs. Considering the variations in aquatic environments under different conditions, the uranium capture capacity of piezoelectric catalysis was examined under the circumstance of 100 ppm uranium at pH 2-8 (Supplementary Fig. 16). Remarkably, ZnO@COF-40 displays higher removal efficiency under acidic conditions compared to those of ZnO and COF, maintaining excellent uranium removal efficiency across a broader pH range. This variance may be linked to the restricted carrier recombination at the interface between ZnO and COF after combination, which aids in enhancing piezoelectric catalytic activity. Subsequently, adsorption kinetics experiments were conducted with ZnO@COF-40 to confirm the effectiveness of the piezoelectric material in capturing uranium (Fig. 5b and Supplementary Table 2). Notably, the adsorption capacity of ZnO@COF-40 under ultrasonic treatment reaches up to 2336.74 mg g−1, significantly surpassing the adsorption capacity without ultrasonic treatment (389.67 mg g−1). Under ultrasonic irradiation, a substantial amount of piezoelectric charge is generated in ZnO@COF-40. This leads to the transformation of U(VI) adsorbed on the ZnO@COF-40 surface to insoluble U(IV), facilitating enhanced utilization of active sites and attaining higher uranium adsorption capacity.
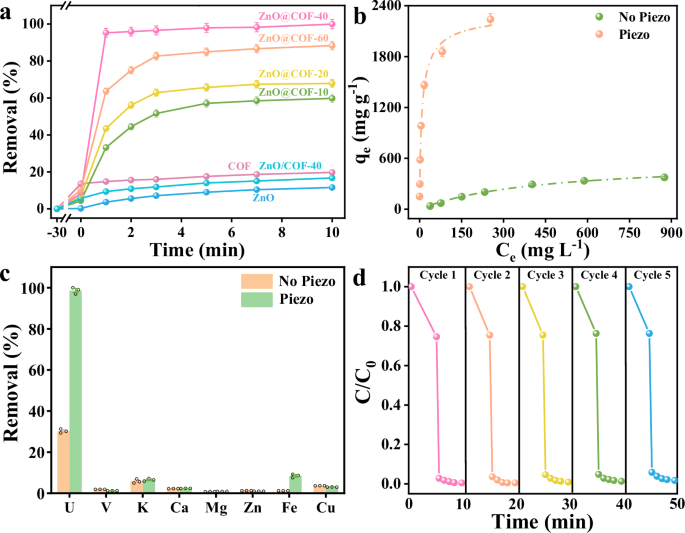
a The piezo catalytic efficiency of U(VI) by ZnO, COF, and ZnO@COF after 10 minutes of ultrasonic treatment (CU(VI) = 100 mg L−1, pH 5). b The adsorption isotherm of UO22+ on ZnO@COF-40 under ultrasonic and without ultrasonic treatment (pH 5). c The uranium removal rate of ZnO@COF-40 in the presence of competing cations containing 10 ppm U. d Recyclability tests of ZnO@COF-40 for U(VI) extraction. Error bars represent S.D. n = 3 independent experiments. Source data for Fig. 5 are available as a Source Data file.
It is widely recognized that various cations and anions coexist with U(VI) in seawater, and the presence of these ions significantly influences the adsorption and migration of the target metal ions. Hence, the selectivity of ZnO@COF-40 for U(VI) via piezoelectric catalysis was examined. As depicted in Fig. 5c and Supplementary Fig. 17, ZnO@COF-40 selectively extracts U(VI) with minimal interference from other competing ions. The excellent selectivity of ZnO@COF-40 for U(VI) is attributed to its abundance of hydroxyl and aldehyde groups capable of forming stable chelates with uranium ions. High extraction efficiency paired with good selectivity allows ZnO@COF-40 to efficiently extract uranium in complex aqueous environments, which is of considerable practical significance. Furthermore, through five consecutive cycles of experimentation, ZnO@COF-40 continues to demonstrate high piezoelectric catalytic efficiency without significant decline in activity (Fig. 5d), confirming its remarkable stability and reusability.
Piezocatalytic reduction of uranium mechanism
The mechanism behind the effective piezoelectric extraction of UO22+ was investigated. As shown in Supplementary Fig. 18, a new distinctive peak (909 cm−1) attributed to O = U = O is observed in the FT-IR spectrum following piezoelectric catalytic reduction of U(VI), indicating the attachment of uranium to the adsorbent18. SEM images reveal numerous spherical crystals and amorphous uranium distributed on the surface of ZnO@COF-40 after ultrasonic treatment (Supplementary Fig. 19). Energy spectrum analysis (EDS) reveals that these spherical crystals are mainly composed of uranium and oxygen elements, indicating the formation of uranium oxides post uranium reduction (Supplementary Fig. 20). Subsequent analysis of the valence states of uranium on ZnO@COF-40 after piezoelectric catalysis was conducted using XPS. In the survey spectra of ZnO@COF-40 + U (Supplementary Fig. 21), distinct U 4 f and U 4 d peaks are observed compared to the sample prior to adsorption. Further examination of high-resolution spectra (Supplementary Fig. 22) reveals the coexistence of both U(VI) and U(IV) in different valence states of uranium. The U 4 d peak is attributed to U3O7, confirming the presence of both U(IV) and U(V) oxidation states46. This indicates the transformation of uranium across various valence states during the uranium extraction process utilizing ZnO@COF-40 piezoelectric catalysis. PXRD experiments were performed to further analyze the uranium oxide obtained after the reaction. As shown in Supplementary Fig. 23, ZnO@COF-40 displays new characteristic peaks at 12.03° and 28.26° following uranium extraction. Upon careful examination, these peaks are identified as UO3•2H2O (PDF 13-0241) and UO2 (PDF 36-0089), consistent with the oxidation states determined by XPS analysis. Therefore, it is speculated that ZnO@COF-40 may first reduce UO22+ to UO2 during the piezoelectric catalytic uranium extraction process. Subsequently, UO2 is quickly oxidized to U3O7 upon exposure to air, and further oxidizes to UO3•2H2O. To validate this hypothesis and further elucidate the mechanism of the piezocatalytic process, several radical scavengers were utilized during the extraction procedure. As illustrated in Supplementary Fig. 24, the presence of p-BQ and DDQ significantly reduces the removal of U(VI), indicating that •O2− and e− are the primary active species in the piezoelectric catalytic process. This phenomenon results from the generation of a substantial amount of h+ and e− by ZnO@COF-40 under ultrasonic irradiation.
(Supplementary Fig. 25). Certain electrons present on the surface of the fabric promptly facilitate the reduction of UO22+ to UO2, while other electrons diminish soluble oxygen to •O2−47. Consequently, •O2− further assists in the reduction of UO22+ to UO2.
To gain a more profound understanding of the interface interactions between ZnO and COF post their composite formation, density functional theory (DFT) calculations have been conducted. To clarify the source of charge transfer at the interface, the work functions (WF) of ZnO and COF have been determined by aligning their Fermi level with the vacuum level (Fig. 6a, b). The WF of ZnO is measured at 5.38 eV, while that of COF registers at 4.87 eV, indicating a higher electrostatic potential of COF compared to ZnO. This results in the establishment of an interface electric field at the ZnO@COF-40 junction (Fig. 6c). Due to the disparity in WF, electrons migrate from COF to ZnO, ultimately decreasing the electron density on the COF side while increasing it on the ZnO side. This, in turn, mitigates the shielding effect of ZnO metallic edge states. Based on this premise, a charge transfer mechanism for the ZnO@COF-40 catalytic reduction of uranium under ultrasonic treatment has been proposed. The formation of a Z-type heterojunction in ZnO@COF-40 eliminates the edge shielding effect of ZnO metallic states and fosters efficient electron transfer throughout the structure. Under mechanical stimulation, ZnO is excited to produce a substantial piezoelectric potential, effectively aiding the separation of free electrons and holes. Simultaneously, the excited electrons in the conduction band (CB) of ZnO are transferred to the valence band (VB) of COF. During the catalytic reduction of uranium, U(VI) in solution is adsorbed onto the active site of COF. Under ultrasonic treatment, electrons in the CB of COF are transferred to the LUMO orbitals of U(VI), resulting in the reduction of U(VI) to U(IV) (Fig. 6d). In summary, the ZnO@COF-40 heterojunction efficiently extracts uranium under simple mechanical stress. This study significantly enhances the comprehension of COF’s role in improving the piezoelectric attributes of two-dimensional materials, offering valuable insights for the design and optimization of piezoelectric catalytic materials.
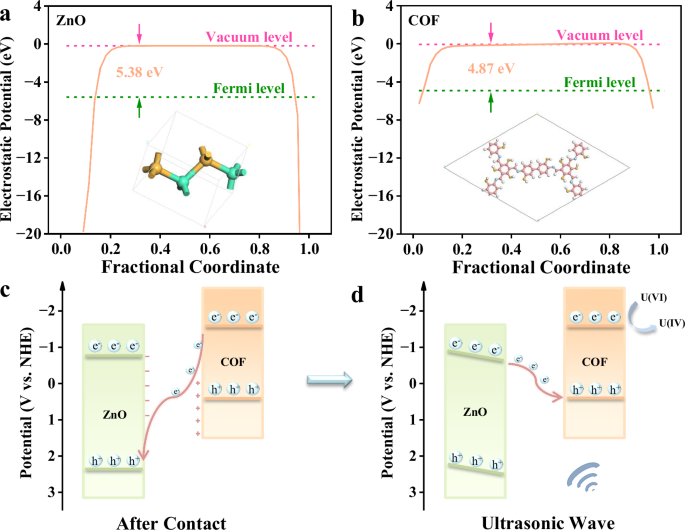
The WF of a ZnO (Zn: green; O: orange) and b COF (C: red; N: light blue; O: orange; H: white) calculated through DFT. Schematic representation of c charge transfer process and d piezocatalytic mechanism of the heterojunction. Source data for Fig. 6 are provided as a Source Data file.
Uranium recovery under simulated ocean waves
Based on the remarkable efficacy of ZnO@COF-40 in the extraction of uranium, research has been conducted on the retrieval of uranium from spiked seawater utilizing ZnO@COF-40. As illustrated in Supplementary Fig. 26, ZnO@COF-40 operates almost as effectively in seawater as it does in pure water. In 10 ppm U spiked seawater, the extraction efficiency of U(VI) by ZnO@COF-40 reaches an impressive 98.53% within 1 minute under ultrasonic conditions. Motivated by these findings, the potential application of ZnO@COF-40 for uranium extraction from natural seawater was further explored. As indicated in Supplementary Fig. 27, the experimental setup simulated a dynamic oceanic environment, generating surface waves at approximately 1.54 cm s−1, slightly lower than the average speed in coastal marine regions (2-3 cm s−1), yet sufficient to replicate the dynamics of actual waves48. Within this simulated setting, ZnO@COF exhibits high selectivity and rapid uptake, achieving a uranium extraction capacity of 37.8 mg g−1 from seawater after 5 days (Supplementary Fig. 28). The average daily uranium extraction capacity is noted at 7.56 mg g−1 d−1, indicating that ZnO@COF-40 can meet the preset target of the UES standard (6 mg g−1) within a single day49. Furthermore, a comprehensive comparison of ZnO@COF-40 with previously reported uranium extraction catalysts and adsorbents demonstrates that its performance ranks at the top of the list (Supplementary Fig. 29 and Supplementary Table 3). ZnO@COF shows extraordinary extraction efficiency and kinetics, which further validates the advantages of piezoelectric catalytic uranium recovery. These findings underscore the potential of ZnO@COF to effectively capture uranium from seawater under simulated low-energy wave conditions and mark the initial successful application of wave energy to recover uranium resources, providing an innovative approach to address the global energy crisis.